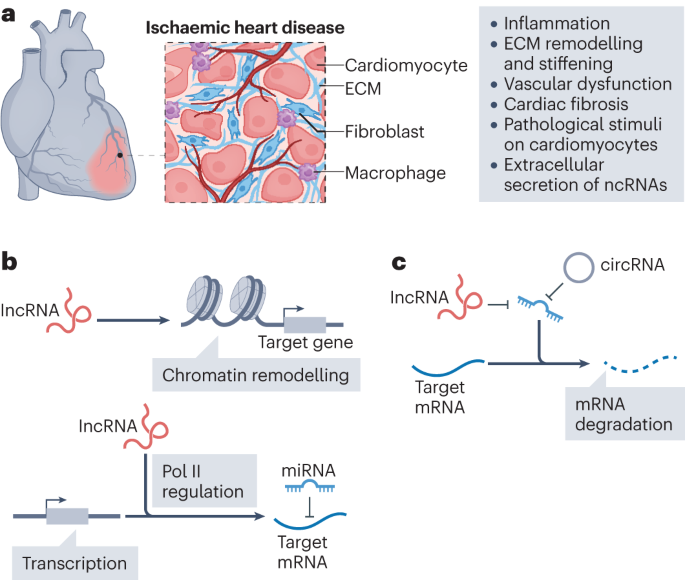
[ad_1]
Berry, C. et al. Small-vessel disease in the heart and brain: current knowledge, unmet therapeutic need, and future directions. J. Am. Heart Assoc. 8, e011104 (2019).
Google ScholarÂ
Mihatov, N., Januzzi, J. L. Jr & Gaggin, H. K. Type 2 myocardial infarction due to supply-demand mismatch. Trends Cardiovasc. Med. 27, 408â417 (2017).
Google ScholarÂ
Thygesen, K. et al. Fourth universal definition of myocardial infarction (2018). J. Am. Coll. Cardiol. 72, 2231â2264 (2018).
Google ScholarÂ
Thygesen, K. et al. Fourth universal definition of myocardial infarction (2018). Eur. Heart J. 40, 237â269 (2019).
Google ScholarÂ
Thygesen, K. et al. Fourth universal definition of myocardial infarction (2018). Circulation 138, e618âe651 (2018).
Google ScholarÂ
Nowbar, A. N., Gitto, M., Howard, J. P., Francis, D. P. & Al-Lamee, R. Mortality from ischemic heart disease. Circ. Cardiovasc. Qual. Outcomes 12, e005375 (2019).
Google ScholarÂ
Taubel, J. et al. Novel antisense therapy targeting microRNA-132 in patients with heart failure: results of a first-in-human phase 1b randomized, double-blind, placebo-controlled study. Eur. Heart J. 42, 178â188 (2021).
Google ScholarÂ
Vedin, O. et al. Significance of ischemic heart disease in patients with heart failure and preserved, midrange, and reduced ejection fraction: a nationwide cohort study. Circ. Heart Fail. 10, e003875 (2017).
Google ScholarÂ
Shah, S. J. et al. Prevalence and correlates of coronary microvascular dysfunction in heart failure with preserved ejection fraction: PROMIS-HFpEF. Eur. Heart J. 39, 3439â3450 (2018).
Google ScholarÂ
John, J. E. et al. Coronary artery disease and heart failure with preserved ejection fraction: the ARIC study. J. Am. Heart Assoc. 11, e021660 (2022).
Google ScholarÂ
Elgendy, I. Y. & Pepine, C. J. Heart failure with preserved ejection fraction: is ischemia due to coronary microvascular dysfunction a mechanistic factor? Am. J. Med. 132, 692â697 (2019).
Google ScholarÂ
Elgendy, I. Y., Mahtta, D. & Pepine, C. J. Medical therapy for heart failure caused by ischemic heart disease. Circ. Res. 124, 1520â1535 (2019).
Google ScholarÂ
Marwick, T. H. Ejection fraction pros and cons: JACC state-of-the-art review. J. Am. Coll. Cardiol. 72, 2360â2379 (2018).
Google ScholarÂ
Chapman, A. R. et al. Long-term outcomes in patients with type 2 myocardial infarction and myocardial injury. Circulation 137, 1236â1245 (2018).
Google ScholarÂ
Berry, C. Stable coronary syndromes: the case for consolidating the nomenclature of stable ischemic heart disease. Circulation 136, 437â439 (2017).
Google ScholarÂ
Reynolds, H. R. et al. Coronary arterial function and disease in women with no obstructive coronary arteries. Circ. Res. 130, 529â551 (2022).
Google ScholarÂ
Herscovici, R. et al. Ischemia and no obstructive coronary artery disease (INOCA): what is the risk? J. Am. Heart Assoc. 7, e008868 (2018).
Google ScholarÂ
Christiansen, M. N. et al. Age-specific trends in incidence, mortality, and comorbidities of heart failure in Denmark, 1995 to 2012. Circulation 135, 1214â1223 (2017).
Google ScholarÂ
Srivaratharajah, K. et al. Reduced myocardial flow in heart failure patients with preserved ejection fraction. Circ. Heart Fail. 9, e002562 (2016).
Google ScholarÂ
Tsao, C. W. et al. Heart disease and stroke statistics-2022 update: a report from the American Heart Association. Circulation 145, e153âe639 (2022).
Google ScholarÂ
Foreman, K. J. et al. Forecasting life expectancy, years of life lost, and all-cause and cause-specific mortality for 250 causes of death: reference and alternative scenarios for 2016 â 40 for 195 countries and territories. Lancet 392, 2052â2090 (2018).
Google ScholarÂ
Andersson, C. & Vasan, R. S. Epidemiology of heart failure with preserved ejection fraction. Heart Fail. Clin. 10, 377â388 (2014).
Google ScholarÂ
Ashokprabhu, N. D., Quesada, O., Alvarez, Y. R. & Henry, T. D. INOCA/ANOCA: mechanisms and novel treatments. Am. Heart J. 30, 100302 (2023).
Schirone, L. et al. An overview of the molecular mechanisms associated with myocardial ischemic injury: state of the art and translational perspectives. Cells 11, 1165 (2022).
Google ScholarÂ
Das, S. et al. Noncoding RNAs in cardiovascular disease: current knowledge, tools and technologies for investigation, and future directions: a scientific statement from the American Heart Association. Circ. Genom. Precis. Med. 13, e000062 (2020).
Google ScholarÂ
Santovito, D. & Weber, C. Non-canonical features of microRNAs: paradigms emerging from cardiovascular disease. Nat. Rev. Cardiol. 19, 620â638 (2022).
Google ScholarÂ
Frantz, S., Hundertmark, M. J., Schulz-Menger, J., Bengel, F. M. & Bauersachs, J. Left ventricular remodelling post-myocardial infarction: pathophysiology, imaging, and novel therapies. Eur. Heart J. 43, 2549â2561 (2022).
Google ScholarÂ
Yan, Y. et al. The cardiac translational landscape reveals that micropeptides are new players involved in cardiomyocyte hypertrophy. Mol. Ther. 29, 2253â2267 (2021).
Google ScholarÂ
Spencer, H. L. et al. The LINC00961 transcript and its encoded micropeptide, small regulatory polypeptide of amino acid response, regulate endothelial cell function. Cardiovasc. Res. 116, 1981â1994 (2020).
Google ScholarÂ
Jonas, S. & Izaurralde, E. Towards a molecular understanding of microRNA-mediated gene silencing. Nat. Rev. Genet. 16, 421â433 (2015).
Google ScholarÂ
Zhong, N., Nong, X., Diao, J. & Yang, G. piRNA-6426 increases DNMT3B-mediated SOAT1 methylation and improves heart failure. Aging 14, 2678â2694 (2022).
Google ScholarÂ
Gao, X. Q. et al. The piRNA CHAPIR regulates cardiac hypertrophy by controlling METTL3-dependent N6-methyladenosine methylation of Parp10 mRNA. Nat. Cell Biol. 22, 1319â1331 (2020).
Google ScholarÂ
Rajan, K. S. et al. Abundant and altered expression of PIWI-interacting RNAs during cardiac hypertrophy. Heart Lung Circ. 25, 1013â1020 (2016).
Google ScholarÂ
Sun, Y. H., Lee, B. & Li, X. Z. The birth of piRNAs: how mammalian piRNAs are produced, originated, and evolved. Mamm. Genome 33, 293â311 (2022).
Google ScholarÂ
Kufel, J. & Grzechnik, P. Small nucleolar RNAs tell a different tale. Trends Genet. 35, 104â117 (2019).
Google ScholarÂ
van Ingen, E. et al. C/D box snoRNA SNORD113-6 guides 2â²-O-methylation and protects against site-specific fragmentation of tRNALeu(TAA) in vascular remodeling. Mol. Ther. Nucleic Acids 30, 162â172 (2022).
Google ScholarÂ
Brameier, M., Herwig, A., Reinhardt, R., Walter, L. & Gruber, J. Human box C/D snoRNAs with miRNA like functions: expanding the range of regulatory RNAs. Nucleic Acids Res. 39, 675â686 (2011).
Google ScholarÂ
Jagielski, N. P., Rai, A. K., Rajan, K. S., Mangal, V. & Garikipati, V. N. S. A contemporary review of snoRNAs in cardiovascular health: RNA modification and beyond. Mol. Ther. Nucleic Acids 35, 102087 (2024).
Google ScholarÂ
Valkov, N. & Das, S. Y RNAs: biogenesis, function and implications for the cardiovascular system. Adv. Exp. Med. Biol. 1229, 327â342 (2020).
Google ScholarÂ
Li, X. et al. Extracellular vesicle-encapsulated adeno-associated viruses for therapeutic gene delivery to the heart. Circulation 148, 405â425 (2023).
Google ScholarÂ
Kuhle, B., Chen, Q. & Schimmel, P. tRNA renovatio: rebirth through fragmentation. Mol. Cell 83, 3953â3971 (2023).
Google ScholarÂ
Li, Q. et al. tRNA-derived small non-coding RNAs in response to ischemia inhibit angiogenesis. Sci. Rep. 6, 20850 (2016).
Google ScholarÂ
Shen, L. et al. A novel class of tRNA-derived small non-coding RNAs respond to myocardial hypertrophy and contribute to intergenerational inheritance. Biomolecules 8, 54 (2018).
Google ScholarÂ
Nojima, T. & Proudfoot, N. J. Mechanisms of lncRNA biogenesis as revealed by nascent transcriptomics. Nat. Rev. Mol. Cell Biol. 23, 389â406 (2022).
Google ScholarÂ
Li, Y., Syed, J. & Sugiyama, H. RNAâDNA triplex formation by long noncoding RNAs. Cell Chem. Biol. 23, 1325â1333 (2016).
Google ScholarÂ
Niehrs, C. & Luke, B. Regulatory R-loops as facilitators of gene expression and genome stability. Nat. Rev. Mol. Cell Biol. 21, 167â178 (2020).
Google ScholarÂ
Ritter, N. et al. The lncRNA locus handsdown regulates cardiac gene programs and is essential for early mouse development. Dev. Cell 50, 644â657.e8 (2019).
Google ScholarÂ
Balas, M. M. et al. Establishing RNAâRNA interactions remodels lncRNA structure and promotes PRC2 activity. Sci. Adv. 7, eabc9191 (2021).
Google ScholarÂ
Liu, J. et al. LncRNA HBL1 is required for genome-wide PRC2 occupancy and function in cardiogenesis from human pluripotent stem cells. Development 148, dev199628 (2021).
Google ScholarÂ
Yu, J. et al. Long noncoding RNA ahit protects against cardiac hypertrophy through SUZ12 (suppressor of Zeste 12 protein homolog)-mediated downregulation of MEF2A (myocyte enhancer factor 2A). Circ. Heart Fail. 13, e006525 (2020).
Google ScholarÂ
Orom, U. A. et al. Long noncoding RNAs with enhancer-like function in human cells. Cell 143, 46â58 (2010).
Google ScholarÂ
Saldana-Meyer, R. et al. RNA interactions are essential for CTCF-mediated genome organization. Mol. Cell 76, 412â422 (2019).
Google ScholarÂ
Ounzain, S. et al. CARMEN, a human super enhancer-associated long noncoding RNA controlling cardiac specification, differentiation and homeostasis. J. Mol. Cell Cardiol. 89, 98â112 (2015).
Google ScholarÂ
Micheletti, R. et al. The long noncoding RNA Wisper controls cardiac fibrosis and remodeling. Sci. Transl. Med. 9, eaai9118 (2017).
Google ScholarÂ
Plaisance, I. et al. A transposable element into the human long noncoding RNA CARMEN is a switch for cardiac precursor cell specification. Cardiovasc. Res. 119, 1361â1376 (2022).
Google ScholarÂ
Ward, Z., Pearson, J., Schmeier, S., Cameron, V. & Pilbrow, A. Insights into circular RNAs: their biogenesis, detection, and emerging role in cardiovascular disease. RNA Biol. 18, 2055â2072 (2021).
Google ScholarÂ
Barrett, S. P. & Salzman, J. Circular RNAs: analysis, expression and potential functions. Development 143, 1838â1847 (2016).
Google ScholarÂ
Yang, Y. et al. Extensive translation of circular RNAs driven by N6-methyladenosine. Cell Res. 27, 626â641 (2017).
Google ScholarÂ
Li, Z. et al. Exonâintron circular RNAs regulate transcription in the nucleus. Nat. Struct. Mol. Biol. 22, 256â264 (2015).
Google ScholarÂ
Li, X. et al. lncRNA H19 alleviated myocardial I/RI via suppressing miR-877-3p/Bcl-2-mediated mitochondrial apoptosis. Mol. Ther. Nucleic Acids 17, 297â309 (2019).
Google ScholarÂ
Zhu, Y. et al. Overexpression of circRNA SNRK targets miR-103-3p to reduce apoptosis and promote cardiac repair through GSK3β/β-catenin pathway in rats with myocardial infarction. Cell Death Discov. 7, 84 (2021).
Google ScholarÂ
Huang, S. et al. Loss of super-enhancer-regulated circRNA Nfix induces cardiac regeneration after myocardial infarction in adult mice. Circulation 139, 2857â2876 (2019).
Google ScholarÂ
Garikipati, V. N. S. et al. Circular RNA CircFndc3b modulates cardiac repair after myocardial infarction via FUS/VEGF-A axis. Nat. Commun. 10, 4317 (2019).
Google ScholarÂ
Alarcon, C. R., Lee, H., Goodarzi, H., Halberg, N. & Tavazoie, S. F. N6-methyladenosine marks primary microRNAs for processing. Nature 519, 482â485 (2015).
Google ScholarÂ
Chamorro-Jorganes, A. et al. METTL3 regulates angiogenesis by modulating let-7e-5p and miRNA-18a-5p expression in endothelial cells. Arterioscler. Thromb. Vasc. Biol. 41, e325âe337 (2021).
Google ScholarÂ
Li, L. et al. m6A methylation in cardiovascular diseases: from mechanisms to therapeutic potential. Front. Genet. 13, 908976 (2022).
Google ScholarÂ
Wu, J. et al. N6-methyladenosine modification opens a new chapter in circular RNA biology. Front. Cell Dev. Biol. 9, 709299 (2021).
Google ScholarÂ
Sweaad, W. K. et al. Relevance of N6-methyladenosine regulators for transcriptome: implications for development and the cardiovascular system. J. Mol. Cell Cardiol. 160, 56â70 (2021).
Google ScholarÂ
Cusenza, V. Y., Tameni, A., Neri, A. & Frazzi, R. The lncRNA epigenetics: the significance of m6A and m5C lncRNA modifications in cancer. Front. Oncol. 13, 1063636 (2023).
Google ScholarÂ
Nishikura, K. A-to-I editing of coding and non-coding RNAs by ADARs. Nat. Rev. Mol. Cell Biol. 17, 83â96 (2016).
Google ScholarÂ
Marceca, G. P. et al. MiREDiBase, a manually curated database of validated and putative editing events in microRNAs. Sci. Data 8, 199 (2021).
Google ScholarÂ
van der Kwast, R. et al. Adenosine-to-inosine editing of vasoactive microRNAs alters their targetome and function in ischemia. Mol. Ther. Nucleic Acids 21, 932â953 (2020).
Google ScholarÂ
Wang, Q. et al. ADAR1 regulates ARHGAP26 gene expression through RNA editing by disrupting miR-30b-3p and miR-573 binding. RNA 19, 1525â1536 (2013).
Google ScholarÂ
Kokot, K. E. et al. Reduction of A-to-I RNA editing in the failing human heart regulates formation of circular RNAs. Basic Res. Cardiol. 117, 32 (2022).
Google ScholarÂ
Vlachogiannis, N. I. et al. Adenosine-to-inosine Alu RNA editing controls the stability of the pro-inflammatory long noncoding RNA NEAT1 in atherosclerotic cardiovascular disease. J. Mol. Cell Cardiol. 160, 111â120 (2021).
Google ScholarÂ
Novikova, I. V., Hennelly, S. P. & Sanbonmatsu, K. Y. Structural architecture of the human long non-coding RNA, steroid receptor RNA activator. Nucleic Acids Res. 40, 5034â5051 (2012).
Google ScholarÂ
Gong, J. et al. LNCediting: a database for functional effects of RNA editing in lncRNAs. Nucleic Acids Res. 45, D79âD84 (2017).
Google ScholarÂ
Athanasiadis, A., Rich, A. & Maas, S. Widespread A-to-I RNA editing of Alu-containing mRNAs in the human transcriptome. PLoS Biol. 2, e391 (2004).
Google ScholarÂ
Hergenreider, E. et al. Atheroprotective communication between endothelial cells and smooth muscle cells through miRNAs. Nat. Cell Biol. 14, 249â256 (2012).
Google ScholarÂ
Caporali, A. et al. p75NTR-dependent activation of NF-κB regulates microRNA-503 transcription and pericyte-endothelial crosstalk in diabetes after limb ischaemia. Nat. Commun. 6, 8024 (2015).
Google ScholarÂ
Chang, Y. J. et al. Extracellular microRNA-92a mediates endothelial cellâmacrophage communication. Arterioscler. Thromb. Vasc. Biol. 39, 2492â2504 (2019).
Google ScholarÂ
Li, J. et al. Mir-30d regulates cardiac remodeling by intracellular and paracrine signaling. Circ. Res. 128, e1âe23 (2021).
Google ScholarÂ
Mathiyalagan, P. et al. Angiogenic mechanisms of human CD34+ stem cell exosomes in the repair of ischemic hindlimb. Circ. Res. 120, 1466â1476 (2017).
Google ScholarÂ
Huang, F. et al. Exosomally derived Y RNA fragment alleviates hypertrophic cardiomyopathy in transgenic mice. Mol. Ther. Nucleic Acids 24, 951â960 (2021).
Google ScholarÂ
Jeppesen, D. K., Zhang, Q., Franklin, J. L. & Coffey, R. J. Extracellular vesicles and nanoparticles: emerging complexities. Trends Cell Biol. 33, 667â681 (2023).
Google ScholarÂ
Emanueli, C. et al. Coronary artery-bypass-graft surgery increases the plasma concentration of exosomes carrying a cargo of cardiac microRNAs: an example of exosome trafficking out of the human heart with potential for cardiac biomarker discovery. PLoS ONE 11, e0154274 (2016).
Google ScholarÂ
He, D. et al. Total internal reflection-based single-vesicle in situ quantitative and stoichiometric analysis of tumor-derived exosomal microRNAs for diagnosis and treatment monitoring. Theranostics 9, 4494â4507 (2019).
Google ScholarÂ
Lee, H. et al. Caveolin-1 selectively regulates microRNA sorting into microvesicles after noxious stimuli. J. Exp. Med. 216, 2202â2220 (2019).
Google ScholarÂ
Cheng, H. et al. Hypoxia-challenged MSC-derived exosomes deliver miR-210 to attenuate post-infarction cardiac apoptosis. Stem Cell Res. Ther. 11, 224 (2020).
Google ScholarÂ
Freeman, D. W. et al. Altered extracellular vesicle concentration, cargo, and function in diabetes. Diabetes 67, 2377â2388 (2018).
Google ScholarÂ
Cable, J. et al. Exosomes, microvesicles, and other extracellular vesicles-a Keystone Symposia report. Ann. N. Y. Acad. Sci. 1523, 24â37 (2023).
Google ScholarÂ
Ben-Aicha, S. et al. High-density lipoprotein remodelled in hypercholesterolaemic blood induce epigenetically driven down-regulation of endothelial HIF-1α expression in a preclinical animal model. Cardiovasc. Res. 116, 1288â1299 (2020).
Google ScholarÂ
Zhang, Q. et al. Supermeres are functional extracellular nanoparticles replete with disease biomarkers and therapeutic targets. Nat. Cell Biol. 23, 1240â1254 (2021).
Google ScholarÂ
Jeppesen, D. K. et al. Reassessment of exosome composition. Cell 177, 428â445 (2019).
Google ScholarÂ
Zhang, Q. et al. Transfer of functional cargo in exomeres. Cell Rep. 27, 940â954 (2019).
Google ScholarÂ
Welsh, J. A. et al. Minimal information for studies of extracellular vesicles (MISEV2023): from basic to advanced approaches. J. Extracell. Vesicles 13, e12404 (2024).
Google ScholarÂ
Davidson, S. M. et al. Methods for the identification and characterization of extracellular vesicles in cardiovascular studies: from exosomes to microvesicles. Cardiovasc. Res. 119, 45â63 (2023).
Google ScholarÂ
Coumans, F. A. W. et al. Methodological guidelines to study extracellular vesicles. Circ. Res. 120, 1632â1648 (2017).
Google ScholarÂ
Thery, C. et al. Minimal information for studies of extracellular vesicles 2018 (MISEV2018): a position statement of the International Society for Extracellular Vesicles and update of the MISEV2014 guidelines. J. Extracell. Vesicles 7, 1535750 (2018).
Google ScholarÂ
exRNA Atlas: data, tools & computable knowledge. https://exrna-atlas.org (US National Instiutes of Health, 2024).
Murillo, O. D. et al. exRNA atlas analysis reveals distinct extracellular rna cargo types and their carriers present across human biofluids. Cell 177, 463â477 (2019).
Google ScholarÂ
Rozowsky, J. et al. exceRpt: a comprehensive analytic platform for extracellular RNA profiling. Cell Syst. 8, 352â357 (2019).
Google ScholarÂ
Fish, J. E. et al. miR-126 regulates angiogenic signaling and vascular integrity. Dev. Cell 15, 272â284 (2008).
Google ScholarÂ
Harris, T. A., Yamakuchi, M., Ferlito, M., Mendell, J. T. & Lowenstein, C. J. MicroRNA-126 regulates endothelial expression of vascular cell adhesion molecule 1. Proc. Natl Acad. Sci. USA 105, 1516â1521 (2008).
Google ScholarÂ
Heymans, S. et al. Macrophage microRNA-155 promotes cardiac hypertrophy and failure. Circulation 128, 1420â1432 (2013).
Google ScholarÂ
Thum, T. et al. MicroRNA-21 contributes to myocardial disease by stimulating MAP kinase signalling in fibroblasts. Nature 456, 980â984 (2008).
Google ScholarÂ
Bang, C. et al. Cardiac fibroblast-derived microRNA passenger strand-enriched exosomes mediate cardiomyocyte hypertrophy. J. Clin. Invest. 124, 2136â2146 (2014).
Google ScholarÂ
Care, A. et al. MicroRNA-133 controls cardiac hypertrophy. Nat. Med. 13, 613â618 (2007).
Google ScholarÂ
Foinquinos, A. et al. Preclinical development of a miR-132 inhibitor for heart failure treatment. Nat. Commun. 11, 633 (2020).
Google ScholarÂ
Ucar, A. et al. The miRNA-212/132 family regulates both cardiac hypertrophy and cardiomyocyte autophagy. Nat. Commun. 3, 1078 (2012).
Google ScholarÂ
Lei, Z. et al. miR-132/212 impairs cardiomyocytes contractility in the failing heart by suppressing SERCA2a. Front. Cardiovasc. Med. 8, 592362 (2021).
Google ScholarÂ
Batkai, S. et al. CDR132L improves systolic and diastolic function in a large animal model of chronic heart failure. Eur. Heart J. 42, 192â201 (2021).
Google ScholarÂ
Anand, S. et al. MicroRNA-132-mediated loss of p120RasGAP activates the endothelium to facilitate pathological angiogenesis. Nat. Med. 16, 909â914 (2010).
Google ScholarÂ
Rawal, S. et al. Down-regulation of proangiogenic microRNA-126 and microRNA-132 are early modulators of diabetic cardiac microangiopathy. Cardiovasc. Res. 113, 90â101 (2017).
Google ScholarÂ
Masson, S. et al. Circulating microRNA-132 levels improve risk prediction for heart failure hospitalization in patients with chronic heart failure. Eur. J. Heart Fail. 20, 78â85 (2018).
Google ScholarÂ
Huang, J. P. et al. Exosomal microRNAs miR-30d-5p and miR-126a-5p are associated with heart failure with preserved ejection fraction in STZ-induced type 1 diabetic rats. Int. J. Mol. Sci. 23, 7514 (2022).
Google ScholarÂ
Bonauer, A. et al. MicroRNA-92a controls angiogenesis and functional recovery of ischemic tissues in mice. Science 324, 1710â1713 (2009).
Google ScholarÂ
Hinkel, R. et al. Inhibition of microRNA-92a protects against ischemia/reperfusion injury in a large-animal model. Circulation 128, 1066â1075 (2013).
Google ScholarÂ
Abplanalp, W. T. et al. Efficiency and target derepression of anti-miR-92a: results of a first in human study. Nucleic Acid Ther. 30, 335â345 (2020).
Google ScholarÂ
Porrello, E. R. et al. MiR-15 family regulates postnatal mitotic arrest of cardiomyocytes. Circ. Res. 109, 670â679 (2011).
Google ScholarÂ
Besnier, M. et al. miR-15a/-16 inhibit angiogenesis by targeting the Tie2 coding sequence: therapeutic potential of a miR-15a/16 decoy system in limb ischemia. Mol. Ther. Nucleic Acids 17, 49â62 (2019).
Google ScholarÂ
Spinetti, G. et al. MicroRNA-15a and microRNA-16 impair human circulating proangiogenic cell functions and are increased in the proangiogenic cells and serum of patients with critical limb ischemia. Circ. Res. 112, 335â346 (2013).
Google ScholarÂ
Yang, Y. et al. MicroRNA-34a plays a key role in cardiac repair and regeneration following myocardial infarction. Circ. Res. 117, 450â459 (2015).
Google ScholarÂ
Huang, W. et al. Loss of microRNA-128 promotes cardiomyocyte proliferation and heart regeneration. Nat. Commun. 9, 700 (2018).
Google ScholarÂ
Chen, J. et al. mir-17-92 cluster is required for and sufficient to induce cardiomyocyte proliferation in postnatal and adult hearts. Circ. Res. 112, 1557â1566 (2013).
Google ScholarÂ
Tian, Y. et al. A microRNAâHippo pathway that promotes cardiomyocyte proliferation and cardiac regeneration in mice. Sci. Transl. Med. 7, 279ra238 (2015).
Google ScholarÂ
Eulalio, A. et al. Functional screening identifies miRNAs inducing cardiac regeneration. Nature 492, 376â381 (2012).
Google ScholarÂ
Gabisonia, K. et al. MicroRNA therapy stimulates uncontrolled cardiac repair after myocardial infarction in pigs. Nature 569, 418â422 (2019).
Google ScholarÂ
Ounzain, S. et al. Genome-wide profiling of the cardiac transcriptome after myocardial infarction identifies novel heart-specific long non-coding RNAs. Eur. Heart J. 36, 353â368 (2015).
Google ScholarÂ
Ballantyne, M. D. et al. Smooth muscle enriched long noncoding RNA (SMILR) regulates cell proliferation. Circulation 133, 2050â2065 (2016).
Google ScholarÂ
Bell, R. D. et al. Identification and initial functional characterization of a human vascular cell-enriched long noncoding RNA. Arterioscler. Thromb. Vasc. Biol. 34, 1249â1259 (2014).
Google ScholarÂ
Martens, C. R., Bansal, S. S. & Accornero, F. Cardiovascular inflammation: RNA takes the lead. J. Mol. Cell Cardiol. 129, 247â256 (2019).
Google ScholarÂ
Zhang, M., Gu, H., Xu, W. & Zhou, X. Down-regulation of lncRNA MALAT1 reduces cardiomyocyte apoptosis and improves left ventricular function in diabetic rats. Int. J. Cardiol. 203, 214â216 (2016).
Google ScholarÂ
Han, P. et al. A long noncoding RNA protects the heart from pathological hypertrophy. Nature 514, 102â106 (2014).
Google ScholarÂ
Wang, K. et al. The long noncoding RNA CHRF regulates cardiac hypertrophy by targeting miR-489. Circ. Res. 114, 1377â1388 (2014).
Google ScholarÂ
Cai, B. et al. The long noncoding RNA CAREL controls cardiac regeneration. J. Am. Coll. Cardiol. 72, 534â550 (2018).
Google ScholarÂ
Ponnusamy, M. et al. Long noncoding RNA CPR (cardiomyocyte proliferation regulator) regulates cardiomyocyte proliferation and cardiac repair. Circulation 139, 2668â2684 (2019).
Google ScholarÂ
Chen, Y. et al. Long non-coding RNA ECRAR triggers post-natal myocardial regeneration by activating ERK1/2 signaling. Mol. Ther. 27, 29â45 (2019).
Google ScholarÂ
Piccoli, M. T. et al. Inhibition of the cardiac fibroblast-enriched lncRNA Meg3 prevents cardiac fibrosis and diastolic dysfunction. Circ. Res. 121, 575â583 (2017).
Google ScholarÂ
Aghagolzadeh, P. et al. Assessment of the cardiac noncoding transcriptome by single-cell RNA sequencing identifies FIXER, a conserved profibrogenic long noncoding RNA. Circulation 148, 778â797 (2023).
Google ScholarÂ
Yuan, Q. et al. CircRNA DICAR as a novel endogenous regulator for diabetic cardiomyopathy and diabetic pyroptosis of cardiomyocytes. Signal Transduct. Target. Ther. 8, 99 (2023).
Google ScholarÂ
Alhamadani, F. et al. Adverse drug reactions and toxicity of the food and drug administration-approved antisense oligonucleotide drugs. Drug Metab. Dispos. 50, 879â887 (2022).
Google ScholarÂ
Khorkova, O. et al. Long non-coding RNA-targeting therapeutics: discovery and development update. Expert Opin. Drug Discov. 18, 1011â1029 (2023).
Google ScholarÂ
Roberts, T. C., Langer, R. & Wood, M. J. A. Advances in oligonucleotide drug delivery. Nat. Rev. Drug Discov. 19, 673â694 (2020).
Google ScholarÂ
Lim, K. R. Q. & Yokota, T. Invention and early history of gapmers. Methods Mol. Biol. 2176, 3â19 (2020).
Google ScholarÂ
Vasquez, G. et al. Site-specific incorporation of 5â²-methyl DNA enhances the therapeutic profile of gapmer ASOs. Nucleic Acids Res. 49, 1828â1839 (2021).
Google ScholarÂ
Li, D., Mastaglia, F. L., Fletcher, S. & Wilton, S. D. Precision medicine through antisense oligonucleotide-mediated exon skipping. Trends Pharmacol. Sci. 39, 982â994 (2018).
Google ScholarÂ
Gramlich, M. et al. Antisense-mediated exon skipping: a therapeutic strategy for titin-based dilated cardiomyopathy. EMBO Mol. Med. 7, 562â576 (2015).
Google ScholarÂ
Plaisance, I. et al. A transposable element into the human long noncoding RNA CARMEN is a switch for cardiac precursor cell specification. Cardiovasc. Res. 119, 1361â1376 (2023).
Google ScholarÂ
Kramer, M. C. et al. Combinatorial control of Drosophila circular RNA expression by intronic repeats, hnRNPs, and SR proteins. Genes Dev. 29, 2168â2182 (2015).
Google ScholarÂ
Liu, X. M., Zhou, J., Mao, Y., Ji, Q. & Qian, S. B. Programmable RNA N6-methyladenosine editing by CRISPRâCas9 conjugates. Nat. Chem. Biol. 15, 865â871 (2019).
Google ScholarÂ
Nose, K., Hidaka, K., Yano, T., Tomita, Y. & Fukuda, M. Short-chain guide RNA for site-directed A-to-I RNA editing. Nucleic Acid Ther. 31, 58â67 (2021).
Google ScholarÂ
Katrekar, D. et al. Efficient in vitro and in vivo RNA editing via recruitment of endogenous ADARs using circular guide RNAs. Nat. Biotechnol. 40, 938â945 (2022).
Google ScholarÂ
Khosravi, H. M. & Jantsch, M. F. Site-directed RNA editing: recent advances and open challenges. RNA Biol. 18, 41â50 (2021).
Google ScholarÂ
Kanelidis, A. J., Premer, C., Lopez, J., Balkan, W. & Hare, J. M. Route of delivery modulates the efficacy of mesenchymal stem cell therapy for myocardial infarction: a meta-analysis of preclinical studies and clinical trials. Circ. Res. 120, 1139â1150 (2017).
Google ScholarÂ
von Degenfeld, G. et al. Selective pressure-regulated retroinfusion of fibroblast growth factor-2 into the coronary vein enhances regional myocardial blood flow and function in pigs with chronic myocardial ischemia. J. Am. Coll. Cardiol. 42, 1120â1128 (2003).
Google ScholarÂ
Mavropoulos, S. A., Yamada, K. P., Sakata, T. & Ishikawa, K. Cardiac gene delivery in large animal models: antegrade techniques. Methods Mol. Biol. 2573, 147â158 (2022).
Google ScholarÂ
Gao, L. et al. Large cardiac muscle patches engineered from human induced-pluripotent stem cell-derived cardiac cells improve recovery from myocardial infarction in swine. Circulation 137, 1712â1730 (2018).
Google ScholarÂ
Kikuchi, K., McDonald, A. D., Sasano, T. & Donahue, J. K. Targeted modification of atrial electrophysiology by homogeneous transmural atrial gene transfer. Circulation 111, 264â270 (2005).
Google ScholarÂ
Kopechek, J. A. et al. Ultrasound and microbubble-targeted delivery of a microRNA inhibitor to the heart suppresses cardiac hypertrophy and preserves cardiac function. Theranostics 9, 7088â7098 (2019).
Google ScholarÂ
Clarke, D. E., Pashuck, E. T., Bertazzo, S., Weaver, J. V. M. & Stevens, M. M. Self-healing, self-assembled beta-sheet peptide-poly(γ-glutamic acid) hybrid hydrogels. J. Am. Chem. Soc. 139, 7250â7255 (2017).
Google ScholarÂ
Yuan, J. et al. Microneedle patch loaded with exosomes containing microRNA-29b prevents cardiac fibrosis after myocardial infarction. Adv. Healthc. Mater. 12, e2202959 (2023).
Google ScholarÂ
Greenberg, B. et al. Calcium upregulation by percutaneous administration of gene therapy in patients with cardiac disease (CUPID 2): a randomised, multinational, double-blind, placebo-controlled, phase 2b trial. Lancet 387, 1178â1186 (2016).
Google ScholarÂ
Katz, M. G., Fargnoli, A. S., Weber, T., Hajjar, R. J. & Bridges, C. R. Use of adeno-associated virus vector for cardiac gene delivery in large-animal surgical models of heart failure. Hum. Gene Ther. Clin. Dev. 28, 157â164 (2017).
Google ScholarÂ
Subramanian, M. et al. RNAi-mediated rheostat for dynamic control of AAV-delivered transgenes. Nat. Commun. 14, 1970 (2023).
Google ScholarÂ
Bozoglu, T. et al. Endothelial retargeting of AAV9 in vivo. Adv. Sci. 9, e2103867 (2022).
Google ScholarÂ
Zhang, H., Zhan, Q., Huang, B., Wang, Y. & Wang, X. AAV-mediated gene therapy: advancing cardiovascular disease treatment. Front. Cardiovasc. Med. 9, 952755 (2022).
Google ScholarÂ
Li, C. & Samulski, R. J. Engineering adeno-associated virus vectors for gene therapy. Nat. Rev. Genet. 21, 255â272 (2020).
Google ScholarÂ
Yue, R. et al. Mesenchymal stem cell-derived exosomal microRNA-182-5p alleviates myocardial ischemia/reperfusion injury by targeting GSDMD in mice. Cell Death Discov. 8, 202 (2022).
Google ScholarÂ
Aggarwal, R. et al. Surgical porcine model of chronic myocardial ischemia treated by exosome-laden collagen patch and off-pump coronary artery bypass graft. J. Vis. Exp. https://doi.org/10.3791/65553 (2023).
Google ScholarÂ
Cheng, G., Zhu, D., Huang, K. & Caranasos, T. G. Minimally invasive delivery of a hydrogel-based exosome patch to prevent heart failure. J. Mol. Cell Cardiol. 169, 113â121 (2022).
Google ScholarÂ
Herrera-Barrera, M. et al. Peptide-guided lipid nanoparticles deliver mRNA to the neural retina of rodents and nonhuman primates. Sci. Adv. 9, eadd4623 (2023).
Google ScholarÂ
Semple, S. C. et al. Rational design of cationic lipids for siRNA delivery. Nat. Biotechnol. 28, 172â176 (2010).
Google ScholarÂ
Naidu, G. S. et al. A combinatorial library of lipid nanoparticles for cell type-specific mRNA delivery. Adv. Sci. 10, e2301929 (2023).
Google ScholarÂ
Hald Albertsen, C. et al. The role of lipid components in lipid nanoparticles for vaccines and gene therapy. Adv. Drug Deliv. Rev. 188, 114416 (2022).
Google ScholarÂ
Rurik, J. G. et al. CAR T cells produced in vivo to treat cardiac injury. Science 375, 91â96 (2022).
Google ScholarÂ
Ge, X., Chen, L., Zhao, B. & Yuan, W. Rationale and application of PEGylated lipid-based system for advanced target delivery of siRNA. Front. Pharmacol. 11, 598175 (2020).
Google ScholarÂ
Scognamiglio, I. et al. Transferrin-conjugated SNALPs encapsulating 2â²-O-methylated miR-34a for the treatment of multiple myeloma. Biomed. Res. Int. 2014, 217365 (2014).
Google ScholarÂ
Lou, J. et al. Reactive oxygen species (ROS) activated liposomal cell delivery using a boronate-caged guanidine lipid. Chemistry 28, e202201057 (2022).
Google ScholarÂ
US National Library of Medicine. ClinicalTrials.gov https://clinicaltrials.gov/study/NCT05350969 (2024).
US National Library of Medicine. ClinicalTrials.gov https://clinicaltrials.gov/study/NCT03603431 (2019).
Biomarkers Definitions Working Group. Biomarkers and surrogate endpoints: preferred definitions and conceptual framework. Clin. Pharmacol. Ther. 69, 89â95 (2001).
Google ScholarÂ
Schulte, C. et al. Serial measurements of protein and microRNA biomarkers to specify myocardial infarction subtypes. J. Mol. Cell Cardiol. 1, 100014 (2022).
DâAlessandra, Y. et al. Circulating microRNAs are new and sensitive biomarkers of myocardial infarction. Eur. Heart J. 31, 2765â2773 (2010).
Google ScholarÂ
Blanco-Dominguez, R. et al. A novel circulating MicroRNA for the detection of acute myocarditis. N. Engl. J. Med. 384, 2014â2027 (2021).
Google ScholarÂ
Koch, C. et al. Nanopore sequencing of DNA-barcoded probes for highly multiplexed detection of microRNA, proteins and small biomarkers. Nat. Nanotechnol. 18, 1483â1491 (2023).
Google ScholarÂ
Almaghrbi, H., Giordo, R., Pintus, G. & Zayed, H. Non-coding RNAs as biomarkers of myocardial infarction. Clin. Chim. Acta 540, 117222 (2023).
Google ScholarÂ
Lalem, T. & Devaux, Y. Circulating microRNAs to predict heart failure after acute myocardial infarction in women. Clin. Biochem. 70, 1â7 (2019).
Google ScholarÂ
Wen, Z. J. et al. Emerging roles of circRNAs in the pathological process of myocardial infarction. Mol. Ther. Nucleic Acids 26, 828â848 (2021).
Google ScholarÂ
Devaux, Y. et al. MicroRNA-150: a novel marker of left ventricular remodeling after acute myocardial infarction. Circ. Cardiovasc. Genet. 6, 290â298 (2013).
Google ScholarÂ
Scrutinio, D., Conserva, F., Guida, P. & Passantino, A. Long-term prognostic potential of microRNA-150-5p in optimally treated heart failure patients with reduced ejection fraction: a pilot study. Minerva Cardiol. Angiol. 70, 439â446 (2022).
Google ScholarÂ
Abu-Halima, M. et al. Micro-RNA 150-5p predicts overt heart failure in patients with univentricular hearts. PLoS ONE 14, e0223606 (2019).
Google ScholarÂ
Lin, X., Zhang, S. & Huo, Z. Serum circulating miR-150 is a predictor of post-acute myocardial infarction heart failure. Int. Heart J. 60, 280â286 (2019).
Google ScholarÂ
Aonuma, T. et al. MiR-150 attenuates maladaptive cardiac remodeling mediated by long noncoding RNA MIAT and directly represses profibrotic Hoxa4. Circ. Heart Fail. 15, e008686 (2022).
Google ScholarÂ
Kawaguchi, S. et al. SPRR1A is a key downstream effector of MiR-150 during both maladaptive cardiac remodeling in mice and human cardiac fibroblast activation. Cell Death Dis. 14, 446 (2023).
Google ScholarÂ
Aonuma, T. et al. Cardiomyocyte microRNA-150 confers cardiac protection and directly represses proapoptotic small proline-rich protein 1A. JCI Insight 6, e150405 (2021).
Google ScholarÂ
Tang, Y. et al. MicroRNA-150 protects the mouse heart from ischaemic injury by regulating cell death. Cardiovasc. Res. 106, 387â397 (2015).
Google ScholarÂ
Li, J. et al. Microvesicle-mediated transfer of microRNA-150 from monocytes to endothelial cells promotes angiogenesis. J. Biol. Chem. 288, 23586â23596 (2013).
Google ScholarÂ
Xiao, J. et al. Circulating miR-30d predicts survival in patients with acute heart failure. Cell. Physiol. Biochem. 41, 865â874 (2017).
Google ScholarÂ
Melman, Y. F. et al. Circulating microRNA-30d is associated with response to cardiac resynchronization therapy in heart failure and regulates cardiomyocyte apoptosis: a translational pilot study. Circulation 131, 2202â2216 (2015).
Google ScholarÂ
Vausort, M. et al. Myocardial infarction-associated circular RNA predicting left ventricular dysfunction. J. Am. Coll. Cardiol. 68, 1247â1248 (2016).
Google ScholarÂ
Salgado-Somoza, A., Zhang, L., Vausort, M. & Devaux, Y. The circular RNA MICRA for risk stratification after myocardial infarction. Int. J. Cardiol. Heart Vasc. 17, 33â36 (2017).
Google ScholarÂ
Posada, D. & Buckley, T. R. Model selection and model averaging in phylogenetics: advantages of akaike information criterion and Bayesian approaches over likelihood ratio tests. Syst. Biol. 53, 793â808 (2004).
Google ScholarÂ
Badimon, L. et al. Cardiovascular RNA markers and artificial intelligence may improve COVID-19 outcome: a position paper from the EU-CardioRNA COST Action CA17129. Cardiovasc. Res. 117, 1823â1840 (2021).
Google ScholarÂ
Firat, H. et al. FIMICS: a panel of long noncoding RNAs for cardiovascular conditions. Heliyon 9, e13087 (2023).
Google ScholarÂ
Sopic, M. et al. Integration of epigenetic regulatory mechanisms in heart failure. Basic Res. Cardiol. 118, 16 (2023).
Google ScholarÂ
Jusic, A., Stellos, K., Ferreira, L., Baker, A. H. & Devaux, Y. (Epi)transcriptomics in cardiovascular and neurological complications of COVID-19. J. Mol. Cell Cardiol. 1, 100013 (2022).
Vausort, M. et al. Regulation of N6-methyladenosine after myocardial infarction. Cells 11, 2271 (2022).
Google ScholarÂ
Robinson, E. L., Emanueli, C., Martelli, F. & Devaux, Y. Leveraging non-coding RNAs to fight cardiovascular disease: the EU-CardioRNA network. Eur. Heart J. 42, 4881â4883 (2021).
Google ScholarÂ
de Gonzalo-Calvo, D. et al. Consensus guidelines for the validation of qRT-PCR assays in clinical research by the CardioRNA consortium. Mol. Ther. Methods Clin. Dev. 24, 171â180 (2022).
Google ScholarÂ
de Gonzalo-Calvo, D., Perez-Boza, J., Curado, J., Devaux, Y. & EU-CardioRNA COST Action CA17129. Challenges of microRNA-based biomarkers in clinical application for cardiovascular diseases. Clin. Transl. Med. 12, e585 (2022).
Google ScholarÂ
de Gonzalo-Calvo, D., Sopic, M., Devaux, Y. & EU-CardioRNA COST Action CA17129. Methodological considerations for circulating long noncoding RNA quantification. Trends Mol. Med. 28, 616â618 (2022).
Google ScholarÂ
Lakkisto, P. et al. Development of circulating microRNA-based biomarkers for medical decision-making: a friendly reminder of what should NOT be done. Crit. Rev. Clin. Lab. Sci. 60, 141â152 (2023).
Google ScholarÂ
Vanhaverbeke, M. et al. Peripheral blood RNA biomarkers for cardiovascular disease from bench to bedside: a position paper from the EU-CardioRNA COST action CA17129. Cardiovasc. Res. 118, 3183â3197 (2022).
Google ScholarÂ
Schuldt, A. Great expectations of small RNAs. Nat. Rev. Mol. Cell Biol. 11, 676 (2010).
Google ScholarÂ
Lee, R. C. & Ambros, V. An extensive class of small RNAs in Caenorhabditis elegans. Science 294, 862â864 (2001).
Google ScholarÂ
Pasquinelli, A. E. et al. Conservation of the sequence and temporal expression of let-7 heterochronic regulatory RNA. Nature 408, 86â89 (2000).
Google ScholarÂ
Thum, T. et al. MicroRNAs in the human heart: a clue to fetal gene reprogramming in heart failure. Circulation 116, 258â267 (2007).
Google ScholarÂ
Hullinger, T. G. et al. Inhibition of miR-15 protects against cardiac ischemic injury. Circ. Res. 110, 71â81 (2012).
Google ScholarÂ
Porrello, E. R. et al. Regulation of neonatal and adult mammalian heart regeneration by the miR-15 family. Proc. Natl Acad. Sci. USA 110, 187â192 (2013).
Google ScholarÂ
Ramanujam, D. et al. MicroRNA-21-dependent macrophage-to-fibroblast signaling determines the cardiac response to pressure overload. Circulation 143, 1513â1525 (2021).
Google ScholarÂ
Qiao, L. et al. microRNA-21-5p dysregulation in exosomes derived from heart failure patients impairs regenerative potential. J. Clin. Invest. 129, 2237â2250 (2019).
Google ScholarÂ
Hinkel, R. et al. AntimiR-21 prevents myocardial dysfunction in a pig model of ischemia/reperfusion injury. J. Am. Coll. Cardiol. 75, 1788â1800 (2020).
Google ScholarÂ
Mayourian, J. et al. Exosomal microRNA-21-5p mediates mesenchymal stem cell paracrine effects on human cardiac tissue contractility. Circ. Res. 122, 933â944 (2018).
Google ScholarÂ
De Rosa, S. et al. Transcoronary concentration gradients of circulating microRNAs in heart failure. Eur. J. Heart Fail. 20, 1000â1010 (2018).
Google ScholarÂ
Galluzzo, A. et al. Identification of novel circulating microRNAs in advanced heart failure by next-generation sequencing. Esc. Heart Fail. 8, 2907â2919 (2021).
Google ScholarÂ
Yan, M. et al. miR-21-3p regulates cardiac hypertrophic response by targeting histone deacetylase-8. Cardiovasc. Res. 105, 340â352 (2015).
Google ScholarÂ
Boxhammer, E. et al. MicroRNA-30d-5p-A potential new therapeutic target for prevention of ischemic cardiomyopathy after myocardial infarction. Cells 12, 2369 (2023).
Google ScholarÂ
Li, J. et al. Targeting miR-30d reverses pathological cardiac hypertrophy. EBioMedicine 81, 104108 (2022).
Google ScholarÂ
Hua, C. C., Liu, X. M., Liang, L. R., Wang, L. F. & Zhong, J. C. Targeting the microRNA-34a as a novel therapeutic strategy for cardiovascular diseases. Front. Cardiovasc. Med. 8, 784044 (2021).
Google ScholarÂ
Matsushima, S. & Sadoshima, J. The role of sirtuins in cardiac disease. Am. J. Physiol. Heart Circ. Physiol. 309, H1375âH1389 (2015).
Google ScholarÂ
Seeger, T. & Boon, R. A. MicroRNAs in cardiovascular ageing. J. Physiol. 594, 2085â2094 (2016).
Google ScholarÂ
Zhu, J. N. et al. Activation of miR-34a-5p/Sirt1/p66shc pathway contributes to doxorubicin-induced cardiotoxicity. Sci. Rep. 7, 11879 (2017).
Google ScholarÂ
Rogg, E. M. et al. Analysis of cell type-specific effects of microRNA-92a provides novel insights into target regulation and mechanism of action. Circulation 138, 2545â2558 (2018).
Google ScholarÂ
Zhu, W. & Li, X. Liquid biopsy in coronary heart disease. Methods Mol. Biol. 2695, 279â293 (2023).
Google ScholarÂ
Jakob, P. et al. Loss of angiomiR-126 and 130a in angiogenic early outgrowth cells from patients with chronic heart failure: role for impaired in vivo neovascularization and cardiac repair capacity. Circulation 126, 2962â2975 (2012).
Google ScholarÂ
Zampetaki, A. et al. Plasma microRNA profiling reveals loss of endothelial miR-126 and other microRNAs in type 2 diabetes. Circ. Res. 107, 810â817 (2010).
Google ScholarÂ
Matkovich, S. J. et al. MicroRNA-133a protects against myocardial fibrosis and modulates electrical repolarization without affecting hypertrophy in pressure-overloaded adult hearts. Circ. Res. 106, 166â175 (2010).
Google ScholarÂ
Hu, S. et al. MicroRNA-210 as a novel therapy for treatment of ischemic heart disease. Circulation 122, S124âS131 (2010).
Google ScholarÂ
Zaccagnini, G. et al. Hypoxia-induced miR-210 modulates the inflammatory response and fibrosis upon acute ischemia. Cell Death Dis. 12, 435 (2021).
Google ScholarÂ
Cambier, L. et al. Y RNA fragment in extracellular vesicles confers cardioprotection via modulation of IL-10 expression and secretion. EMBO Mol. Med. 9, 337â352 (2017).
Google ScholarÂ
Busscher, D., Boon, R. A. & Juni, R. P. The multifaceted actions of the lncRNA H19 in cardiovascular biology and diseases. Clin. Sci. 136, 1157â1178 (2022).
Google ScholarÂ
Boulberdaa, M. et al. A role for the long noncoding RNA SENCR in commitment and function of endothelial cells. Mol. Ther. 24, 978â990 (2016).
Google ScholarÂ
Bhatt, D. L., Lopes, R. D. & Harrington, R. A. Diagnosis and treatment of acute coronary syndromes: a review. JAMA 327, 662â675 (2022).
Google ScholarÂ
Mukherjee, D. Myocardial infarction with nonobstructive coronary arteries: a call for individualized treatment. J. Am. Heart Assoc. 8, e013361 (2019).
Google ScholarÂ
Byrne, R. A. et al. 2023 ESC guidelines for the management of acute coronary syndromes. Eur. Heart J. 44, 3720â3826 (2023).
Google ScholarÂ
[ad_2]
Source link